The MBST magnetic resonance technology explained in detail
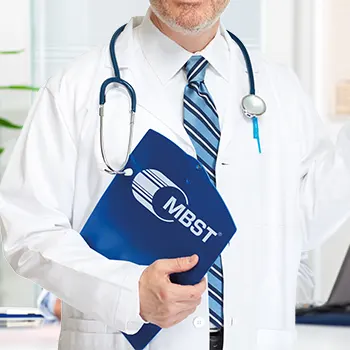
In the following, we want to give a detailed explanation for people with an interest in technology of the therapeutically used MBST magnetic resonance technology, a development based on the physical effect of magnetic resonance that is also used in MRI technology, and show where the similarities and differences lie.
It is important to note that both MRI (magnetic resonance imaging) and MBST magnetic resonance therapy do not expose the human body to any harmful radiation. Instead, MRI devices and MBST therapy devices use magnets and radio signals to influence hydrogen protons in the body in a measurable way. In MRI, these changes can be used to generate images from the inside of the body and to visualise conspicuous structures in the tissue. MBST therapy involves the stimulation of cells and cell groups of a specific type of tissue, with the aim of triggering different processes.
Both procedures are painless and, according to current knowledge, are no burden for the patient. The patient feels nothing of the processes taking place inside his body.
The history of magnetic resonance imaging (MRI)
The physical phenomenon of magnetic resonance was first described in 1946 by Felix Bloch and Edward Purcell. It states that atomic nuclei stimulated by electromagnetic fields are themselves measurable sources of energy. In 1952, they were awarded with the Nobel Prize in Physics for their work.
20 years later, Paul C. Lauterbur and Sir Peter Mansfield were able to show that magnetic resonance can be used to visualize spatial structures. With the help of magnetic resonance imaging, changes and diseases of organs can be gently visualized – without the use of X-rays. However, the actual application of imaging methods in medical diagnostics did not begin until the early 1980s. As magnetic resonance imaging (MRI), the procedure has revolutionized radiological diagnostics. Today, there are more than 60 million MRI scans done worldwide every year.
Functional principle MRI
Put simply, the method makes use of the different distribution of hydrogen atoms in the human body. In a strong magnetic field that surrounds the patient lying in a tube, the normally disordered oscillating atoms are realigned. In this process, they absorb energy. Some of it is released again after the magnetic field has been switched off. These signals are measured and converted into images.
What sounds very simple at first is an extremely complex technology that takes advantage of certain biophysical properties of atomic nuclei.
Atomic nuclei with an odd nucleon number have the property of nuclear spin, i.e. they have an inherent angular momentum (spin).
The simplest atomic nucleus with an odd number of nucleons that generates a magnetic field is the hydrogen nucleus with only one proton.
In the human organism, the hydrogen atom is by far the most common atom, accounting for up to 70% of the body mass.
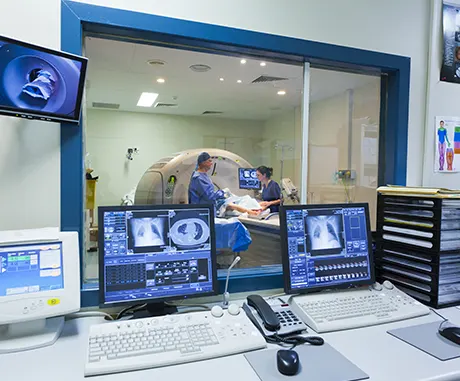
Magnetic field during MRI
Since moving charges induce a magnetic field, the spin of the positively charged hydrogen protons also generates a magnetic field (magnetic dipole moment). In the natural environment (earth’s magnetic field), the atomic nuclei align their magnetic dipole moment in all directions of space. Since the magnetic vectors of the individual atomic nuclei cancel each other out, there is no externally measurable magnetisation. This also applies to the hydrogen atoms of the human body.
By applying a strong external magnetic field, the dipole moments of the hydrogen atoms can be aligned, i.e. if the human body is placed into a strong magnetic field, the magnetic dipole moments will be aligned parallel or antiparallel according to the field lines.
The parallel alignment is energetically slightly more favourable than the antiparallel alignment. Therefore, it occurs more frequently and the dipole moments show a net magnetization (M-vector) in the sum in parallel alignment to the static magnetic field.
What is a magnetic vector in MRI?
The magnetic vector adds up from the parallel aligned magnetic moments of the single hydrogen nuclei and has an angular momentum, which results from the "spins" of the single atoms. This angular momentum corresponds to a gyroscopic motion and is defined as core precession. The individual atoms differ in the frequency of nuclear precession. For hydrogen, ω at 1.5 Tesla is approximately 65 Mhz and is thus in the range of radio waves. This precession frequency is also called Larmor frequency or resonance frequency and is proportional to the strength of the applied magnetic field (B0). If the preceding magnetic (sum) vector is deflected by external influences, the alignment of the vector changes, which can now be measured from the outside.
Note
The Larmor formula describes the interdependencies of these parameters as follows: ωo = γ B0 (γ is the gyromagnetic ratio, a constant proportionality factor specific to each atom).
Phenomenon magnetic resonance
The transfer of energy to the hydrogen nuclei directs them out of their stable rotational position. The deflection can only be achieved by supplying energy from outside. In MRI, this is achieved by short-term irradiation of a high-frequency radio pulse – pulsed magnetic resonance – which has the precession frequency of the hydrogen atoms.
Relaxation time
The magnetic vector follows the precession movement of the nuclei in its alignment and is deflected to different degrees depending on the duration of the pulse. When the pulse is switched off, the rotating magnetic vector tends to return to its original low-energy state in parallel with the external magnetic field. However, the nuclear spin system can only release the energy absorbed by the radiated radio pulse by transmitting it to its environment, i.e. the spin lattice or directly adjacent nuclei. The so-called T1 relaxation time measures this coupling of the nuclear moments to their lattice environment (syn.: spin lattice relaxation time or longitudinal relaxation time). The T2 relaxation time (syn.: spin-spin relaxation time, transversal relaxation time) expresses the strength of the coupling of the magnetic core moments with each other.
Note
Different tissues have different T1 and T2 relaxation times and different proton densities. In order to produce high-contrast images and be able to make the different tissues visible, the magnetic resonance tomograph has to take images with different settings. Depending on the representation, the images are referred to as T1, T2 or proton density weighted images. For example, different tissues can only be clearly distinguished from each other on the basis of their different fat or water content. On T1-weighted images, fat and bone marrow are light, internal organs and body fluids and bones dark. On T2-weighted images liquids are light and fat and bone dark. On proton dense images fat appears light and liquids dark.
MRI sequences
The intensity of the magnetic resonance signal at a certain point in time is influenced by the applied field strength and other parameters. The influence of the individual parameters on the signal can be weighted differently by the structure of the measurement sequence. Tissue-specific differences in relaxation behavior can be leveled or highlighted. By means of special coding processes, the signal can be related to the location of its origin and assigned spatially. The intensity of the measured signal is expressed as the brightness value of a pixel (voxel).
The performance of magnets in MRI · Tesla
The magnetic resonance tomograph is a very large device and contains a very strong, cylindrical magnet. Modern clinically used magnetic resonance tomographs usually have magnets with a field strength of 1.5 or 3 Tesla and sometimes already 7 Tesla and more (ultra-high field MRI). Higher Tesla values improve the device’s accuracy and the resolution as more hydrogen protons can be detected.
Note
In this context it is mportant that MRI must address all hydrogen protons in all tissue types in order to produce a sharp, high-contrast image. MBST therapy, on the other hand, only addresses one specific type of tissue and therefore does not require such high magnetic strength, but rather the precisely matching field strength to stimulate the hydrogen protons in the target tissue.
Differences between MRI and MBST therapy!
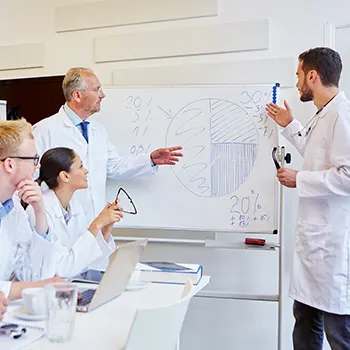
How do I get a good MRI image and what influence does this have on MBST therapy?
An MRI image of the foot shows various details very clearly, such as the ankle joint, the tibia bone, the calf muscles, the Achilles tendon or the skin. This level of detail is achieved by a variety of MRI sequences and exact weighting of the images to highlight tissue-specific features/differences for diagnostic purposes.
Note
In MBST therapy, the impulse sequences are used to influence a specific tissue. The MBST magnetic resonance technology provides the tissue to be treated with energy that triggers processes.
Magnetic field strength, gradients and the resulting loud knocking noises
A magnetic resonance tomograph is very large and requires a specially shielded room. In order to prevent a negative influence on the image quality, no other radio waves or electrical devices may interfere with the examination.
The MRI machine does not expose the patient to a uniformly strong magnetic field. It's stronger on one side of the body, weaker on the other. This results in a magnetic field gradient, i.e. only a thin layer of protons is detected. If this layer is irradiated with a radio wave of the corresponding Larmor frequency, only these protons are stimulated and only signals from the receiving coil are registered.
MRI technology generates further gradients at right angles to the recording plane. These are subdivided into columns and rows and can be read like a table. Every time these gradient fields are switched on and off, the so-called "Lorentz forces" act on the suspensions of the coils, causing the MRI-typical knocking noises.
Note
The required magnetic strengths and electrical energies for MBST therapy do not have to be as strong as they need to be for MRI. The MBST therapy device is therefore almost noiseless during operation.
Note
The MBST spin technology uses the adiabatic fast passage. This is a method for reversing nuclear spin orientations and a prerequisite for magnetic resonance. WIKIPEDIA The generation of repeated spin resonance sequences according to the principle of adiabatic fast passage allows the generation of magnetic spin resonance conditions in weak magnetic fields. This means that the magnetic resonance condition can also be used for small and medium-sized systems. The procedure is used in magnetic resonance therapy. Three interacting magnetic fields are required for magnetic resonance therapy: first, a static main magnetic field, second, a modulated magnetic field parallel to it, and third, an alternating field that fulfills the Larmor condition and is perpendicular to the other two. The magnetic field strength of the modulated magnetic field then passes around the static field while the frequency remains constant. When the strength is lowered, the alternating field is also activated. The aim is to correlate the frequency of the modulated magnetic field with the spin lattice relaxation time. The typical magnetic field is generated in a Helmholtz coil.
MRI · Claustrophobia and need to keep still for a long time
For an MRI scan, the patients are usually positioned in a narrow tube and must lie as still as possible for the duration of the examination. Moving can cause the resulting images to become blurred, so that the examination must be repeated. In this case, the patient must remain in the MRI for a longer period of time. Patients with claustrophobia or other anxieties therefore often take sedatives for the examination.
Note
The MBST therapy devices have an open design, allowing the patient to move freely within the treatment area during treatment. Since the target is not an image that is as as sharp as possible, it is not necessary to lie completely still. During the treatment, the patient can relax on the lying surface and, for example, read, listen to music or even sleep.